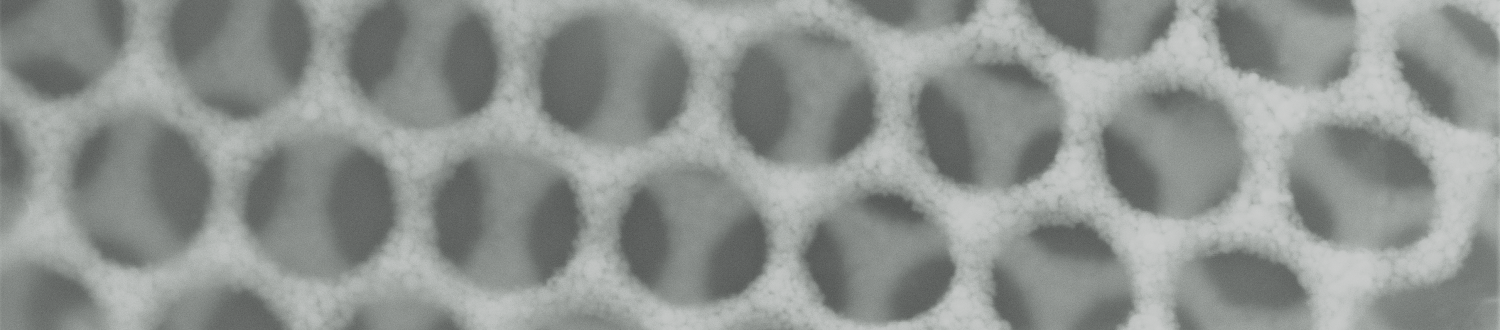
Biological physics
Biological physics is a multidisciplinary field that explores biological systems with a physicists' mindset. While biological physics investigates similar objects with biochemistry and molecular biology, biological physics can be characterised by using advanced tools such as high-resolution fluorescence imaging, electron microscopy, atomic force microscopy and concepts such as force, entropy and information processing which often require knowledge of physics.
Research leads
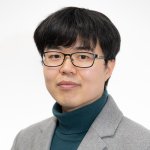
Dr Wooli Bae
Lecturer in Experimental Soft Matter Physics
Dr Isabella Guido
Senior Lecturer in Experimental Biological Physics
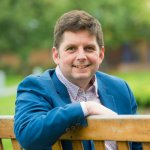
Professor Joseph Keddie
Professor of Soft Matter Physics and Royal Society Industry Fellow
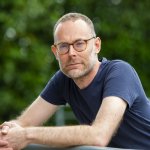
Dr Richard Sear
Associate Professor (aka Reader), Soft Matter, Biological and Medical Physics Group leader, Sustainability Fellow with the Institute for Sustainability
Overview
In the group we integrate principles from physics, chemistry and biology into our work to understand the behaviour and function of living matter at different scale by collaborating with other research groups. Our topics range from living organisms such as bacteria to subcellular systems such as bioinspired active networks of cytoskeletal biopolymers, protocells and DNA-based nanosystems. Our research utilises microfluidics, microscopy, and various molecular biology techniques. We work closely with theorists to formulate predictive theories in the field of active matter physics and bottom-up synthetic biology.
Development of active bio-inspired systems
Cells are considered the basic units of living organisms, but at first glance this representation fails to capture the complexity of a single cell. In fact, the cellular processes that sustain life are the result of intricate networks of molecular interactions. Reducing the complexity of those cellular or subcellular processes to a tractable set of basic building blocks is a fascinating experimental challenge. It will not only improve our understanding of the fundamental principles underlying those processes, but can also lead to synthetic bioinspired systems able to mimic natural structures. The development of such dynamic and out-of-equilibrium structures to understand self-organisation in biological matter is the goal of this interdisciplinary research project, which lies at the intersection of active matter physics and synthetic biology. We assembled active structures at single filament level to develop beating structures similar to cilia and flagella, and at multifilament level to analyse pattern formation in cytoskeletal systems.
Bio-inspired beating systems: https://doi.org/10.1021/acs.nanolett.9b01091
Pattern formation in active network: https://doi.org/10.1021/acs.nanolett.0c01546.
NANOPC (EPSRC)
Cells, the basic units of life, are dynamic systems with continuously operating circuits that sense the environment and adapt to it through a variety of responses. Understanding and engineering living cells, however, is greatly challenging, due to the complexity of the
biochemical networks that underlie a cell's most sophisticated functions. A simpler synthetic alternative to real cells – protocells – can therefore be built to design systems with cell-like traits. Here, we are developing a Nucleic Acid Nanotechnology Originated ProtoCell (NANOPC) system using rationally designed DNA and RNA components. State-of-the-art DNA/RNA nanotechnology is being used to build nanostructures and reaction circuits that mimic proteins and genetic networks of a cell.
HT-PHAC (BBSRC)
Synthetic biology engineers living systems to perform useful functions. For example, small bacteria are engineered to produce expensive vitamins or to degrade plastic wastes. However, cells do not behave same even when their genetic information is same. For example, when we engineer a cell to produce a specific molecule, some cells produce it efficiently while other cells do not. This is a problem because the overall yield of production is reduced. The increase of the production cost is one of the major obstacles that needs to be overcome to commercialise a lot of synthetic biology applications. However, it is difficult to understand why cells show different responses due to their complexity. Even a simple bacteria cell has more than one million molecules inside. In this proposal, we will develop a simple cell mimic – artificial cell system made from scratch using synthetic elements – to understand why cells show different responses despite sharing same genetic information. Using our simple cell mimic, we will gain insight on why cells show different behaviours. A microfluidic device will be used to produce artificial cells at a large scale enough to analyse different populations. The result will be analysed using a mathematical model to understand role of different components in a cell. This knowledge will allow us to engineer cells in a way that they show homogeneous and consistent behaviour. Therefore, this work will help commercialising a lot of synthetic biology applications by reducing their production costs.
NEO (Horizon Europe)
Humankind is producing and storing data at an unprecedented rate, with all known data worldwide expected to grow to 250 Zettabytes by 2025. Unfortunately, all current archival storage media face fundamental limitations that threaten our ability to even store all this data. Hard disk drives suffer from well-known scaling issues, while tape drives suffer from media obsolescence, as data stored on the tape has to be continuously migrated to deal with device failures and technology upgrades, making it a costly storage medium and producing substantial electronic waste. In brief, existing storage technology is too costly while also leading to a considerable negative impact on the environment. If we are to preserve even just a fraction of the world’s data, we are in desperate need of a radically new storage media with substantially better information density, durability, and less carbon footprints. DNA has been posited as a novel storage media thanks to its benefits, such as durability and density, among others. By building on biotechnology, DNA storage is also future proof: DNA synthesis and sequencing will always be available and even get cheaper. Virtually all research (and commercial) efforts on DNA storage today focus on oligo-based DNA storage, i.e., data is encoded and stored in sequential DNA molecules. The major bottleneck of the oligo-based approach, however, is the cost and speed of DNA synthesis, which is required to write each bit (more precisely, roughly 1.6 bits can be written through the addition of one nucleotide to the molecule). We believe that a radically different approach is needed to make DNA storage a reality. In particular, we need an approach that does not rely on DNA synthesis to write data. Our vision, therefore, is to use DNA nanostructures with molecular bumps to store data—in a similar manner to a compact disc—and to use microscopy, followed by a decoding pipeline based on computer vision and machine learning, to reconstruct the original data.
Airborne transmission of viruses, eg flu and COVID
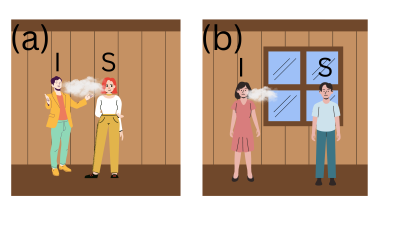
We have worked on understanding and modelling the transmission of airborne diseases such as COVID and flu. An aim has been to understand and predict how Non-Pharmaceutical Interventions (NPIs) such as mask wearing and improved room ventilation reduce transmission.
tl;dr: FFP2 masks and similar (eg N95) should reduce the amount of airborne virus you inhale by about 90% (when worn sensibly). Surgical and cloth masks are much less effective than FFP2s. So if you want to mask, wear an FFP2 or similar. More details are available on the project website.
We are also working to understand how viruses survive drying out. There is good evidence that COVID-19 can be transmitted across a room, but to do so the virus must survive drying.