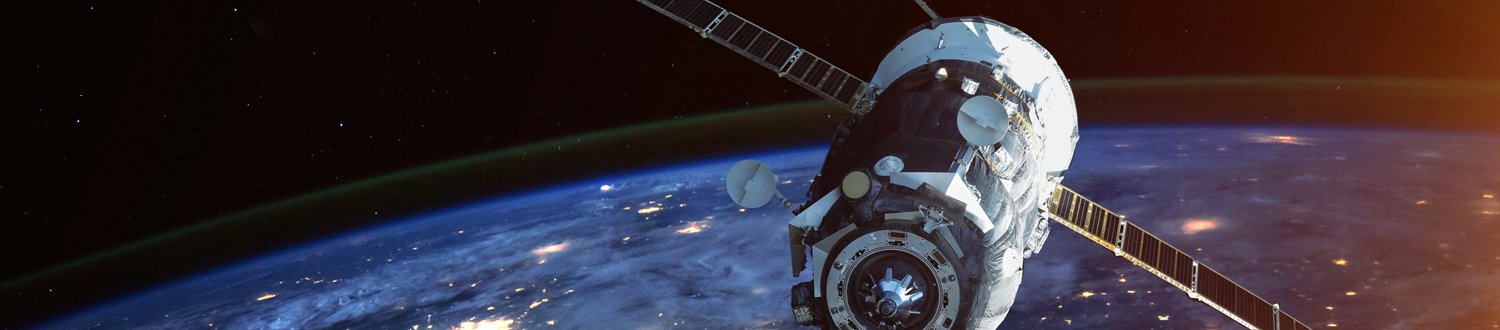
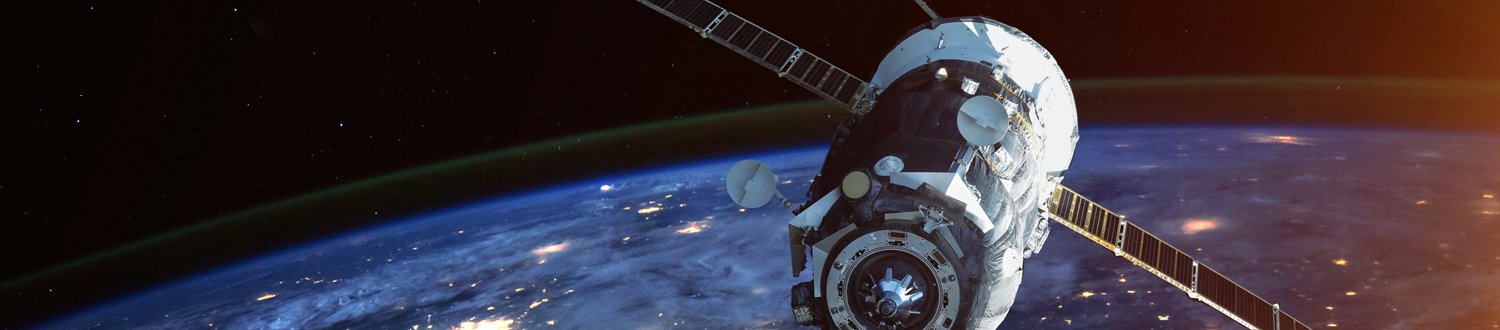
CubeSail
CubeSail is an exciting, ground-breaking educational satellite project we have. A key feature is the deployment of a 25 square metre sail structure, which will be used to demonstrate the propulsive effect of solar radiation pressure (i.e. solar sailing) and will demonstrate the de-orbiting capabilities of the sail as a drag augmentation device.
Overview
CubeSail will be the first launched three-axis stabilised solar sail, and makes use of a novel centre of mass/centre of pressure offset technique to provide enhanced attitude control.
CubeSail will build on our small satellite experience, such as the STRaND-1 nanosatellite, launched on 25 February 2013. Furthermore, the mission critical sail deployment mechanism has undergone an extensive testing and validation process as part of the ESA Gossamer Deorbiter project carried out here.
Several PhD projects are centred on Cubesail's development, and it will serve as a technology platform for at least two further educational satellites developed here. The CubeSail mission objectives are ambitious, and will raise the technology readiness level (TRL) of several technologies to flight demonstration level.
CubeSail satellite
In its launch configuration, the CubeSail satellite conforms to the 3U CubeSat specifications, and the satellite structure has been designed to maximize the additional space provided by the ISIPOD CubeSat deployer. In particular, the available cylindrical space at the rear of the ISIPOD is fully utilised to house a momentum wheel, a sun sensor and deployable boom supporting the magnetometer. The satellite bus occupies approximately 1U, and the remaining 2U house the two-axis translation stage and sail-deployment subsystem.
In the deployed configuration a number of devices will be deployed:
- First, the antennas of the UHF/VHF transceiver are released to enable communication with the CubeSail satellite. These tape-spring antennas are positioned at the base of the bus.
- Next, a 400mm long bi-stable carbon composite boom is deployed from the cylindrical hub at the base of the satellite, which supports a magnetometer required for attitude control during the de-tumbling operation phase.
- After the satellite rotation has stabilised, triggering a burn wire releases four 3U sized solar panels, which are hinged at the base of the satellite bus. The panels rotate by 90 degrees to lie in a plane perpendicular to the satellite (and parallel to the deployed sail).
- Lastly, a pin-puller releases the sail deployment mechanism, and four 3.6m long metallic tape spring booms will be deployed using a motor drive, pulling along the four quadrants of the 5x5m aluminised Kapton solar sail. The sail deployment subsystem is a crucial and mechanically complex subsystem of CubeSail.
An extensive testing and validation campaign as part of ESA's ARTES 5.1 programme under the "Deployable Gossamer Sail for Deorbiting" project (ESA contract number 4000103499/11/NL/US) has raised the TRL of the sail deployment subsystem to TRL5, making it ready for a flight demonstration mission.
Objectives
The primary objectives for the CubeSail mission are twofold. First, to demonstrate the concept of solar sail propulsion in LEO with a 3-axis stabilised 25 square metre solar sail and second, to demonstrate the use of gossamer structures as a drag augmentation device for satellite deorbiting.
The CubeSail mission therefore consists of three stages:
During the commissioning stage, the 3U CubeSat will be de-tumbled using magnetorquers and a momentum wheel. After the rotation of the spacecraft has stabilised, the 25m2 sail structure is deployed.
The CubeSail mission will demonstrate the deployment of the largest gossamer structure deployed from a CubeSat to date.
Over a period of one year, the propulsive effect of solar radiation pressure (SRP) in LEO is demonstrated. In order to minimise drag effects, the sail plane is expected to lie in the direction of travel. Thus, the SRP will result in an orbit inclination drift, rather than an altitude change.
To successfully demonstrate its solar sailing capabilities, CubeSail requires three-axis attitude control. Two axes are controlled by means of a centre of mass/centre of pressure offset technique, complemented by three magnetorquers and a momentum wheel to provide full three-axis attitude control.
While interplanetary solar sailing was successfully demonstrated in the JAXA IKAROS mission, CubeSail will be the first mission to demonstrate solar sailing in low earth orbit, and the first mission to demonstrate full three-axis attitude control of a solar sail.
After the solar sail demonstration stage, CubeSail will be rotated by 90 degrees to align the sail plane perpendicular to the direction of travel. At altitudes of approximately 600km, aerodynamic drag will dominate the SRP, and the large surface area of the sail will result in a rapid decay of the satellite orbit.
CubeSail will be the first mission to demonstrate LEO deorbiting capabilities of large deployable gossamer structures.
Outputs
- February 2013: "Fly Your Satellite" - CubeSail bids on ESA's "Fly Your Satellite" programme
- June 2010: 2nd International Symposium on Solar Sailing - CubeSail presented at the 2nd International Symposium on Solar Sailing, New York
- March 2010: CubeSail mission on BBC news.
- November 2013: Flight readiness review
- July 2013: Test results review
- March 2013: Test readiness review
- September 2012: Critical design review
- February 2012: Preliminary design review
- June 2011: Concept design review
- January 2011: Project kick-off
- October 2009: Funding awarded.
This project provided the expertise for several FP7 funded projects on deployable space structures, notably DeorbitSail and DEPLOYTECH, as well as ESA research grants and collaboration with industrial partners such as RolaTube and Astrium.
In return, CubeSail benefits from much of the heritage developed through these projects. For example, several of its critical subsystems have been validated under ESA's "Deployable Gossamer Sail for Deorbiting" project, raising the TRL to flight demonstration level; and several of the team members have been actively involved in the STRaND-1 development, developing skills and expertise that are directly transferable to CubeSail.
The flight heritage gained through CubeSail will form the basis of further CubeSat projects, including demonstration missions for DeorbitSail and InflateSail (a flagship demonstration mission on the QB50 launch, as part of DEPLOYTECH).
In summary, CubeSail serves as an educational and development platform for several CubeSat projects in our Centre, and will help advance the state of the art of small satellite technology in Europe, and affirm the status of our Centre as a world leader in developing innovative solutions for small satellites.
Demonstrations
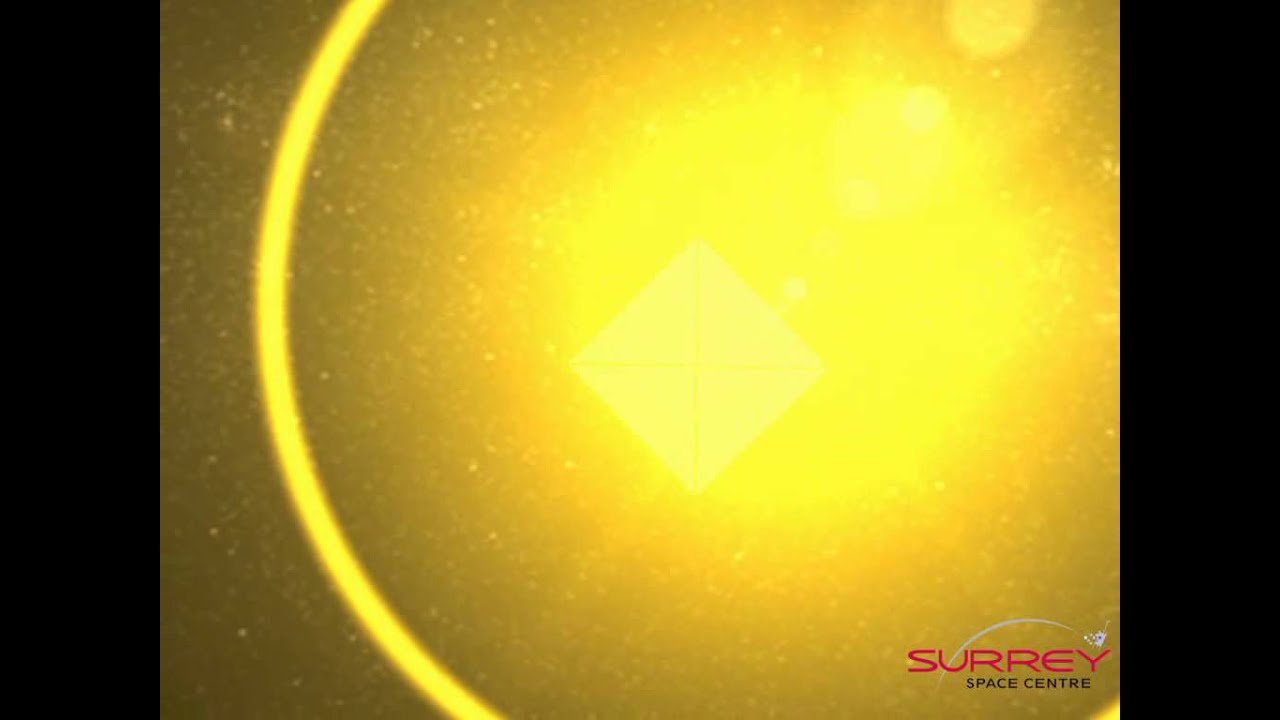
CubeSail flyby
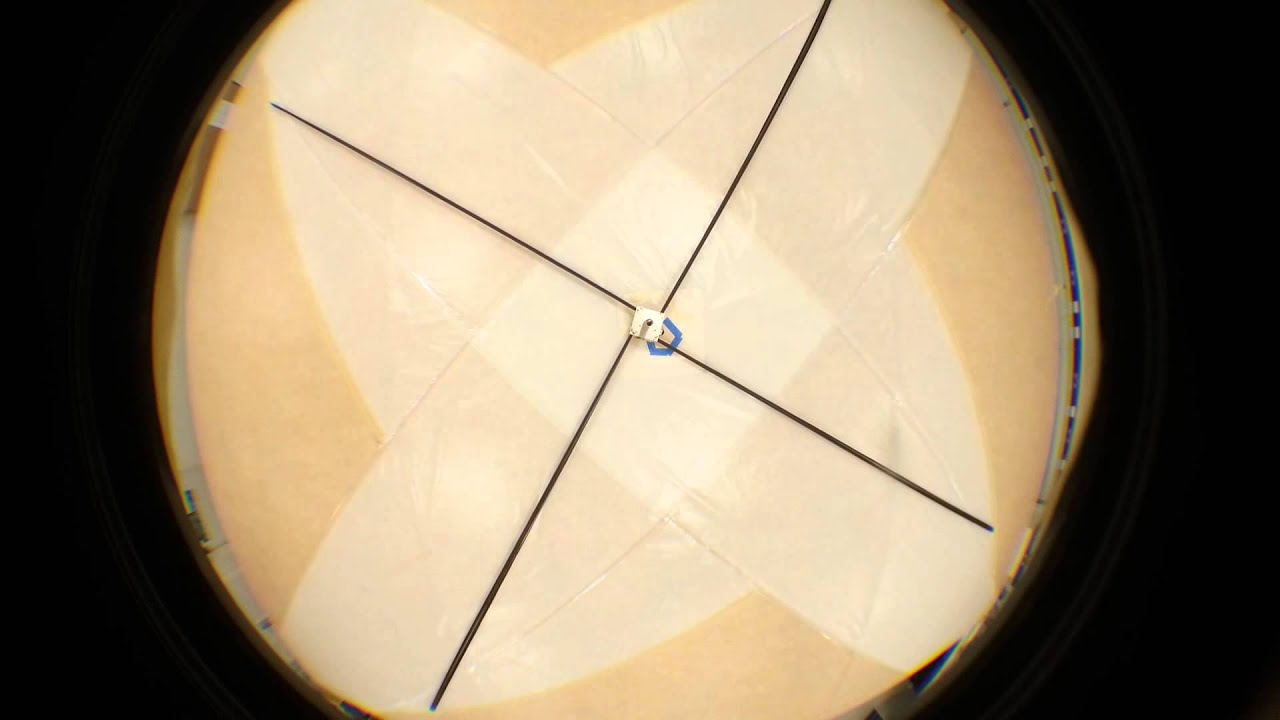
Bistable carbon fiber reinforced polymer booms for 5mx5m solar sail
History of CubeSail technology
Read scientific and historical backgrounds to the types of technologies involved in CubeSail.
The design of a suitable packaging and deployment method for the membrane is an important aspect of the CubeSail and InflateSail projects. The selection of a folding pattern will be strongly affected by the overall design of the de-orbiting device. Existing concepts for the deployment of large space membranes can be divided into the following categories:
Centrifugal deployment and stabilisation
The centrifugal forces provide controlled deployment and stability of the sail against the solar radiation pressure. An important benefit is the scalability of the design, as no rigid components are used. This topology is favoured by Japanese researchers, and the IKAROS launched by JAXA successfully used a spinning central hub to deploy a square solar sail.
Sail supported by diagonal rigid booms
The most common topology for solar sails and de-orbiting devices is a square membrane attached to diagonal rigid booms. The sail suspension method determines the membrane tension state, the loads on the booms and at the satellite attachment point. A brief overview of different designs and their respective advantages is given by Fernandez et al. (2011) and Murphy and Murphy (2002).
Square sails
Both the four- and five-point suspension methods of square membranes have generally been discarded for simpler designs, and no recent projects make use of this concept.
Individual quadrants
This is the generally preferred embodiment for ease of membrane packaging and deployment simplicity (e.g., NanoSail-D, CubeSail, ODISSEE). However, their high slenderness makes the booms sensitive to buckling under the tip loading, which affects the scalability of the design.
Stripped designs
In these designs the membrane is attached at multiple points along the booms; this distributes both the axial and bending (due to solar pressure) loads along the booms, thus improving scalability. The NASA-ISP design by L'Garde uses a "net/membrane stripped" configuration, and Fernandez et al. (2011) describes a "completely stripped" configuration where the membrane is divided into separate narrow strips of sail.
Externally supported polygonal membrane
Inflatable polygonal designs have been used for inflatable antennas (for example by ILC Dover), or investigated for arrays of modular hexagonal membranes (Katsumata et al., 2009).
This section provides a brief overview of existing (proposals for) membrane folding patterns for space applications, and highlights some design considerations.
Wrapping patterns
One method for packaging membranes is by wrapping the membrane around a central hub, by folding along spiralling crease lines. An important benefit is the deployment from the centre outwards. A number of in-extensional wrapping patterns was introduced by Guest and Pellegrino (1992), and further geometries were described by Nojima (2003). It is noted that the thickness of the sheet material can be taken into account numerically when designing the wrapping pattern, thereby optimising the packaging efficiency. Nojima (2007) shows examples of plane tessellation of wrapped polygons; very large sails could therefore be packaged in sections before being joined in the stowed state.
Many such wrapping patterns are designed to be deployed under centrifugal forces. The deployment dynamics were studied by Okuizumi and Yamamoto (2009), both experimentally and with a numerical simulation using a simple spring-mass system. The wrapping patterns may also incorporate a Miura-like folding scheme to reduce the height of the wrapped cylinder, at the expense of complexity (Scheel, 1974).
Leaf folding patterns
The folding pattern used for the IKAROS flight is similar to that described by de Focatiis and Guest (2002) and consists of a flattened version of the "folded hyperbolic paraboloid" which collapses to a star-like configuration. The radial "arms" can subsequently either be z-folded, rolled up or wrapped; the deployment dynamics were analysed by Tibert and Gardsback (2006).
The IKAROS deployment took place in two stages. First the arms were unfurled quasi-statically; the deployment was initiated by the centrifugal forces acting on a small mass at each of the tips, and controlled by a motor drive. The second stage consisted of dynamic deployment of the sail, by releasing the membrane between the arms. One dynamic analysis is given by Okuizumi et al. (2011).
Triangular quadrant folding
In most existing NASA/ESA solar sail designs, the sail consists of four triangular quadrants suspended between deployable booms. This design greatly simplifies the packaging issue, as the boundary conditions on the individual quadrants are relaxed.
Storage
- "Frog leg" folding
- The folding patterns are generally very straightforward. A pattern that resurfaces in various ESA funded projects, is the "Frog Leg" pattern (Vedova et al., 2011). It was successfully demonstrated by the ODISEE project (Leipold et al., 2003). The "double accordion" folding means that in (rigid origami) theory the pattern cannot be deployed in two directions simultaneously.
- Folding and wrapping
- In several solar sail projects, the membrane quadrants are first simply folded, and subsequently wrapped around a single central hub (e.g. for CubeSail, NanoSail-D) or four individual hubs (e.g. for Gossamer-I, NASA-ISP by ATK Engineering). The 20x20m NASA-ISP solar sail designed by ATK employs a similar packaging method; here the booms are deployed first, and the sail is drawn out centrally between two booms and gradually spread wider. This is intended to avoid any possibility for snagging during deployment; the sequenced unfolding is achieved using tabs and tie strips (Murphy et al., 2005).
Deployment
The deployment of the membrane and the booms can either be sequential or simultaneous. The former requires a more complex control procedure, but ensures sufficient boom stiffness before any compressive or bending loads are exerted by the unfurling sail.
- Sequential
- In the ODISEE project, the booms are deployed first, followed by the sail which is unfurled using a rope mechanism (Leipold et al., 1999).
- Simultaneous
- In other designs the booms and sails are deployed simultaneously, e.g. for for NanoSail-D, "The sail membranes ... are z-folded and rolled onto a sail spool. The stored strain energy of the rolled booms provides the driving force to simultaneously deploy both the booms and the sail quadrants." (Johnson et al., 2011).
The deployment process can further either be quasi-static or dynamic:
- Quasi-static
- The quasi-static deployment process has the benefit of imparting minimal impulse to the main satellite, but requires more control. For sequentially deployed systems, the sail deployment will generally be quasi-static. Examples include ODISSEE, and the NASA-ISP solar sail design by L'Garde.
- Dynamic
- The dynamic processes have the benefit of simplicity, and are often driven by the release of strain energy stored in the stowed configuration. Examples include CubeSail and NanoSail-D.
Storage method | Deployment method | |||||
Fold and wrap | "Frog leg" | Sequential | Simultaneous | Dynamic | Quasi-static | |
NanoSail-D | 1 | - | - | X | X | - |
CubeSail | 1 | - | - | X | < 1.5s | - |
ODISSEE | - | X | X | - | - | X |
Gossamer-I | 4 | - | X | - | - | - |
NASA-ISP: ATK | 4 | - | X | - | - | 1cm/sec |
NASA-ISP: L'Garde | - | - | - | X | - | X |
Table 1. Storage and deployment characteristics of several solar sail designs with rigid booms.
Stripped sail configuration
The strippped/striped sail design was first proposed by Greschik and Mikulas (2002) with as motivating factor the distributed loading on the supporting booms, whereas Murphy and Murphy (2002) comment on an increase in mass and complexity due to the increased number of attachment points.
The stowage and deployment is challenging as the membrane is suspended from multiple points along the length of the boom. An implementation was the NASA-ISP design by L'Garde, but the membrane folding method was not specified. The stripped sail concept was extended by Fernandez et al. (2011), who introduced a "completely stripped" sail design, where individual membrane strips are attached to the booms. These are simply z-folded from either end to form two bundles.
Miscellaneous concepts
- Embedded Inflatable Tubes
- Katsumata et al. (2009) describes a wrapped membrane, with embedded inflatable tubes. The inflation accelerates the deployment, and the tubes could impart stiffness to the membrane. Inflation of different tube folding methods is studied by Katsumata et al. (2011).
- Miura-ori folding patterns
- Surprisingly little information is available on the use of the Miura-ori folding pattern to membrane packaging, as proposed by Miura (1985). A manufacturing method suitable for Miura-folding very large scale membranes was developed by Horner et al. (2003).
General comments and issues
- Rigid foldability
- The use of rigid foldable patterns would allow packaging of the membranes with minimal strain energy and material deformation. The question is whether the deployment of thin membranes can be described effectively by the rigid folding kinematics. Existing fold patterns for membrane stowage are generally not rigid foldable.
- Sequential unfolding
- It may be desirable to introduce (passive) methods for controlling the unfolding process; for example, the NASA-ISP design by ATK is contains tabs to delay the unfolding of the membrane to avoid entangling with the booms.
- Deployment loads
- During quasi-static unfurling of the membranes, the solar pressure and drag loading can have a significant impact on the mechanics of the partly deployed structure.
- Premature deployment
- Any residual air stored in the folded membrane may cause premature unfolding; this should be minimised in the packing process, and venting possibilities should be provided in the packaging method (Widani, 2007).
- Structural wrinkles
- The folding pattern may introduce permanent wrinkles in the tensioned membranes, i.e. structural wrinkles, which may be undesirable for certain applications. The effect of the Miura-ori pattern on membrane tensioning was investigated by Papa and Pellegrino (2008).
References
- Dalla Vedova F, Henrion H, Leipold M, Girot T, Vaudemont R, Belmonte T, Fleury K and Couls OL (2011), "The Solar Sail Materials (SSM) project - Status of activities", Advances in Space Research. Vol. 48(11), pp. 1922-1926.
- Fernandez JM, Lappas VJ and Daton-Lovett AJ (2011), "Completely stripped solar sail concept using bi-stable reeled composite booms", Acta Astronautica. Vol. 69(1-2), pp. 78-85.
- de Focatiis DSA and Guest SD (2002), "Deployable membranes designed from folding tree leaves", Philosophical Transactions of the Royal Society A. Vol. 360(1791), pp. 227-238.
- Greschik G and Mikulas MM (2002), "Design Study of a Square Solar Sail Architecture", Journal of Spacecraft and Rockets. Vol. 39(5), pp. 653-661.
- Guest SD and Pellegrino S (1992), "Inextensional Wrapping of Flat Membranes", In First International Conference on Structural Morphology, Montpellier., 7-11 September, 1992. , pp. 203-215.
- Horner G, Wright T and Laue G (2003), "Miura-Ori Solar Sail Packaging Concept Development and Deployment Demonstration", In 39th AIAA/ASME/SAE/ASEE Joint Propulsion Conference and Exhibit. Huntsville, Alabama, July 20-23, 2003. (AIAA-2003-4663)
- Johnson L, Young R, Montgomery E and Alhorn D (2011), "Status of solar sail technology within NASA", Advances in Space Research. Vol. 48(11), pp. 1687-1694.
- Katsumata N, Fujii R, Natori M and Yamakawa H (2009), "Membrane Space Structure Models with Inflatable Tubes", In 27th International Symposium on Space Technology and Science. Tsukuba, Japan, July 5-12, 2009. (2009 c-34)
- Katsumata N, Natori MC and Yamakawa H (2011), "Folding and Deployment Analyses of Inflatable Structures", In The 28th International Symposium on Space Technology and Science. Okinawa, Japan, June 5-12, 2011. (2011-c-38)
- Lappas V, Adeli N, Visagie L, Fernandez J, Theodorou T, Steyn W and Perren M (2011), "CubeSail: A low cost CubeSat based solar sail demonstration mission", Advances in Space Research. Vol. 48(11), pp. 1890-1901.
- Leipold M, Eiden M, Garner C, Herbeck L, Kassing D, Niederstadt T, Krüger T, Pagel G, Rezazad M, Rozemeijer H, Seboldt W, Schöppinger C, Sickinger C and Unckenbold W (2003), "Solar sail technology development and demonstration", Acta Astronautica. Vol. 62(2-6), pp. 317-326.
- Leipold M, Garner C, Freeland R, Hermann A, Noca M, Pagel G, Seboldt W, Sprague G and Unckenbold W (1999), "ODISSEE — A proposal for demonstration of a solar sail in earth orbit", Acta Astronautica. Vol. 45(4-9), pp. 557-566.
- Miura K (1985), "Method of Packaging and Deployment of Large Membranes in Space", The Institute of Space and Astronautical Science report. Vol. 618, pp. 1-9. Japan Aerospace Exploration Agency.
- Murphy DM, McEachen ME, Macy BD and Gaspar JL (2005), "Demonstration of a 20-m Solar Sail System", In 46th AIAA/ASME/ASCE/AHS/ASC Structures, Structural Dynamics & Materials Conference 18 - 21 April 2005, Austin, Texas.
- Murphy DM and Murphy TW (2002), "Scalable Solar Sail Subsystem Design Considerations", In Proceedings of the 43rd AIAA Structures, Structural Dynamics and Materials Conference., 22-25 April, 2002. (AIAA 2002-1703)
- Nojima T (2003), "Modelling of Compact Folding/Wrapping of Flat Circular Membranes (Folding Patterns of Equiangular Spirals)", JSME International Journal Series C. Vol. 46(4), pp. 1547-1553.
- Nojima T (2007), "Origami Modeling of Functional Structures based on Organic Patterns"
- Okuizumi N, Muta A and Matsunaga S (2011), "Enhancement of a Spring-mass System Model for Numerical Simulations of Centrifugal Deployment Dynamics of Folded Square Membranes", In 28th International Symposium on Space Technology and Science. Okinawa, Japan, June 5-12, 2011. (2011-c-30)
- Okuizumi N and Yamamoto T (2009), "Centrifugal Deployment of Membrane with Spiral Folding: Experiment and Simulation", Journal of Space Engineering. Vol. 2(1), pp. 41-50.
- Papa A and Pellegrino S (2008), "Systematically Creased Thin-Film Membrane Structures", Journal of Spacecraft and Rockets. Vol. 45(1), pp. 10-18.
- Scheel HW (1974), "Space-Saving Storage of Flexible Sheets", United States Patent 3848821.
- Tibert G and Gärdsback M (2006), "Space Webs" (ACT-RPT-MAD-ARI-05-4109)
- Widani C (2007), "Solar Sail Deployment Technology and Mechanism", In 1st International Symposium on Solar Sailing.
Solar sail propulsion enables a new range of orbits and missions that would be otherwise inconceivable or tremendously propellant consuming. Some of the major solar sail missions proposed over the years are:
GeoStorm
A mission that will control harmful solar events like corona mass ejects and solar storms and warn in advance. Positioning a solar sail between the Earth and the Sun at approximately 0.98AU can provide two to three times faster warnings than using a satellite at the L1 point (0.993AU).
GeoSail
A low cost mission to study the Earth's magneto-tail. A conventional spacecraft would only have a three month per year window for observation, nevertheless, a solar sail would enter the magneto-tail in each orbit. The European Space Agency (ESA )has conducted several reference studies of this mission and it has been determined that even a low performance solar sail, achievable with the current state of technology, would be suitable for the mission.
Solar Polar Orbiter/Imager
A highly Keplerian orbit mission, only enabled by the use of a large solar sail. The mission involves studying the polar regions of the Sun, thus providing more knowledge of its structure and dynamics.
Solar Sail Kinetic Energy Impactor (KEI)
A mission that deals with the Earth current asteroid threat. It involves several solar sails that would impact the asteroid and consequently change its path. Many missions using conventional spacecraft have been proposed for a similar manner, but the advantage of using solar sails for this objective is that they can achieve higher velocities and subsequently larger momentum transfer to the asteroid.
Interstellar Heliopause Probe (IHP)
A mission to the outer limits of the solar system to investigate the physics of the heliosphere and the local interstellar medium. The solar sail would first approach the Sun, so as to gain the largest possible acceleration, and then, once it gets the energy boost, achieve a hyperbolic trajectory towards the heliopause. The sail would reach distances of up to 200AU in 15-25 years, far beyond the current positions of Voyager 1 and 2. Given the sizes of the solar sail required, it can be classified as a long-term accessible mission.
To enable the feasibility of the missions listed above, solar sails technology readiness level needs to be further increased. For this, simpler demonstration missions are required that will prove that the risky and critical subsystems of deployment and attitude control are reliable enough to be used on more complex scientific missions. The demonstration missions presented next are the past and current attempts at launching a number of solar sails.
Cosmos-1
The launch of Cosmos-1 in 2005 was the first ever attempt of launching a solar sail. However, according to the Planetary Society, the launcher vehicle exploded before the spacecraft could reach orbit. The sail was designed to increase the orbit of the spacecraft around the Earth proving the concept of solar sailing. The spinning disk shaped solar sail had 8x15m blades that could rotate about a single pitch axis for attitude control.
NanoSail-D (1 and 2)
It was the culmination of a decade of research regarding solar sail technology at NASA. In the summer of 2008 NanoSail-D1 was launched aboard a Falcon-1, which unfortunately experienced a failed stage separation and exploded. NASA flew their spare flight model in December 2010. NanoSail-D2 did not have any means of active control, so the several month mission was only intended to demonstrate sail deployment and drag de-orbiting. The sailcraft utilized a 3U cubesat platform, and had a mass of 3kg for a 3.1x3.1m four-quadrant sail.
IKAROS-1
It was launched on May 2010 by the Japanese Space Agency (JAXA). It is the first successful solar sail mission, currently on its way to Venus. A few weeks after the launch, deployment and measurements of the sail characteristic acceleration were taken. IKAROS-1 has a mass of 300 kg and is a 14x14m spinning solar sail. It used the centripetal force generated by its angular rate (spinning) to deploy the sail and produce gyroscopic rigidity for attitude stabilization. For attitude control it uses a novel approached, based on a set of LCDs embedded into the membrane that can change the reflectivity of the sail at different points.
LightSail-1
It is the first of three future projects of the Planetary Society. LightSail-1 is a modification of NanoSail-D that aims to demonstrate deployment of a four-quadrant 32m2 sail with the use of four 4m novel booms developed by the AFRL, called TRAC booms. It is expected to be launched in late 2013 with the mission to demonstrate deployment of the sail in low-Earth orbit (LEO). If LightSail-1 proves successful, LightSail-2 will aim to demonstrate solar sail propulsion, and LightSail-3 will travel to the Sun-Earth L1 point.
Gossamer
It is part of a devised road map for solar sailing proposed by the German Space Agency (DLR) in collaboration with the European Space Agency. In 2014, the one week mission Gossamer-1 is intended to demonstrate deployment of a 5x5m sail with lenticular carbon fiber booms patented by DLR. A year later, Gossamer-2 will deploy a 20x20m sail at a 500 km orbit having active attitude control systems as well. Finally in 2018, Gossamer-3 will deploy a 50x50m sail in medium-Earth orbit (ME0), and after three months, it will escape Earth's orbit using the generated acceleration.
CU Aerospace's CubeSail
CU Aerospace together with the University of Illinois are designing a mission to demonstrate deployment and measure the thrust on a 0:77x260m membrane (around 200m2). This membrane will be deployed from two 1.5U cubesats that will separate from each other in orbit. It is intended as a first step towards a larger sail concept called UltraSail. This last consists of multiple CubeSail-like structures that extend kilometers long film blades to ultimately form an heliogyro.
Drag sails
Space sails can be used to increase the area of the spacecraft that interacts with the atmospheric particles. This increases drag and result in a more rapid de-orbiting. The size of the sail required to successfully deorbit a satellite within a given number of years will depend on the mass of the spacecraft and its initial altitude (atmospheric densities).
The figure above shows de-orbiting times for satellites of up to 500 kg with a 5-by-5-metre deployed sail and optimal pointing control. Satellites that would otherwise remain in orbit for decades beyond the required 25-year lifetime could be brought down quickly with this low-mass, low-power system.
In Earth orbit, drag pressure exceeds solar radiation pressure below approximately 600 km altitude.
Solar sails
For sails orbiting at altitudes above 600 km, the predominant external force will actually be radiation pressure from the sun.
"Solar sailing" is a method of propulsion that uses the very small force exerted by sunlight to propel a spacecraft. This force, solar radiation pressure, is much smaller than the weight of the sail on the earth's surface, even though the sail material is thinner than human hair.
Efficient use of solar radiation pressure requires a very large, very light sail, and also for the sail to be pointed in the correct direction. Because the sail must be very large, it had to be folded for launch and automatically deployed in space. Testing this deployment on Earth can be difficult because the force of gravity is so much larger than the forces the sail has been designed for. One of the challenges is combining computer simulations with experiments to be certain that the sail will deploy in space as it did during laboratory testing. Another challenge is supporting the sail during ground testing of deployment.
Each of the 3.6m long sail support booms consist of two Copper-Beryllium (BeCu) tape springs, facing each other to form a lenticular cross-section, and encased in a Kapton sheath. In this configuration the tape springs are able to slide with respect to each other, significantly reducing the material strains when coiling the booms around the central hub and thus improving packaging efficiency, while still benefiting from an increased bending and torsional rigidity in the deployed state.
The BeCu tape springs are manufactured at SSC, and the spiral-wrapped Kapton sleeves are COTS, manufactured to custom specifications. An aluminium coated version of the Kapton sheath is being considered for thermal control purposes.
The mechanical properties of the metallic booms were characterised for the ESA Gossamer Deorbiter project, including the effects of stress relaxation in long term storage in the coiled configuration [3-D6a]. The BeCu tape springs that form the lenticular-shaped booms have a radius of curvature of 32 mm and subtend an angle of 90 degrees.
Therefore, in the stowed configuration, the packaged height of the booms is approximately 26mm, accounting for the thickness of the outer Kapton sheath. The total thickness of the boom is of about 0.3 mm including the sheath. Each of the four 3.6m long booms has a mass of 162g, for a total of approximately 650g.
The four BeCu booms are attached to and co-coiled around a 32mm diameter spindle, with a combined stored diameter of 87mm. In the stowed configuration, a significant amount of strain energy is stored in the booms, and to avoid 'blossoming' during stowage, the booms are restrained radially using spring-loaded rollers.
During launch the rotation of the spindle is locked, to avoid self-deployment due to vibrations. After releasing the mechanism using a pin-puller (COTS from TiNi Aerospace) the deployment is controlled by rotating the spindle with a brushless DC motor. As the booms uncoil, they are guided through low-friction PTFE rollers to ensure straight deployment. Deployment of the 3.6m booms is expected to take approximately 70s.
The total height of the boom deployer is 75 mm, including the two compartments top and bottom for the springs and the pin-puller.
In 1873 the Scottish physicist James Clark Maxwell theoretically proved the existence of light pressure on "A treatise on electricity and magnetism". Thirty years later, the Russian physicist Peter Lebedew proved it experimentally. But it was the Soviet father of astronautics, Konstantin Tsiolkovsky, in 1936 who suggested the use of solar radiation pressure as a means for space propulsion. In 1951 an American aeronautical engineer named Carl Wiley published the first article in the popular literature regarding "the clipper ships of space". Seven years later, a professor at Columbia University, Richard Garwin, authored the first technical paper and coined the term "solar sailing".
In the early 1970's the development of the space shuttle promised the means to transport and deploy large payloads in Earth orbit. In addition, the development of technologies for deployable space structures and thin films suggested that solar sailing could be considered for a specific mission. In 1973 the Jet Propulsion Laboratory (JPL) proposed the first ever solar sail mission, a rendezvous with comet Halley at its perihelion. Nevertheless, the mission was canceled due to the high risk of deploying the 800x800m suggested sail.
Finally, after forty years of proposed missions that never flew and numerous ground-tested prototypes, in May of 2010, the Japanese Space Agency (JAXA) launched the first solar sail mission, IKAROS, to Venus, demonstrating the power of the Sun for in-space propulsion. Also, solar radiation pressure has been proved useful for controlling the attitude of large GEO satellites using their solar panels, like the Mariner IV.
The attitude control of a sailcraft has a primary and significant role. Since solar sails are continuously accelerating their orbits a quite different from the usual ballistic orbits, followed by conventional spacecraft. The sun angle as has an effect on the direction of the force vector. By choosing a negative or positive sail pitch angle the solar sail can be made to spiral inwards towards the Sun or outwards away from the Sun. The optimal angle for maximising the transverse component is 35.26 degrees so this is often the selected desired pitch orientation for an interplanetary sailcraft in a sun-centred orbit. As such, constant precise attitude control is imperative for the completion of any mission using solar sails.
Another important concept in Solar Sails is the centre of mass (CM) and the centre of pressure (CP). Centre of mass is the mean location of all the mass in a system and can be considered a point where the system will react the same to external forces. Centre of pressure is a point on a body where the sum of all the pressures act causing a force. A Solar Sail with non ideal membrane is statically stable when the CM is between the CP and the sun. When the sail rotates away from its neutral sun pointing position a restoring torque is generated. The solar sails dynamic behaviour is like a pendulum or analogous to that of gravity-gradient stabilised satellite. If the CM lies behind the CP then the sail is unstable and behaves like an inverted pendulum. Most of the research for performing attitude control on Solar Sails concentrates around utilising the restoring force generated by offsetting either the CM or the CP.
Due to manufacturing errors and deployment issues there will always be a CP/CM offset which always causes a disturbance torque on the sailcraft, trying to align it in a specific direction. This offset is usually assumed to be 0.25 per cent.
Spin stabilisation
Spin stabilisation is the simplest method and the most cost effective to control the attitude of any spacecraft. The spacecraft is usually spun around the axis with the largest principal inertia. In the case of solar sail this axis is normal to the sail plane. This spin dynamically stabilises the sail due to gyroscopic stiffness and effectively nullifies any disturbances around the other two axis, as such the sailcraft can maintain its desired orientation in the presence of a CM/CM offset.
A number of different methods have been employed in past conceptual studies. Cosmos 1 used discrete tilted vanes to construct the sail generating a windmill effect. Both Team encounter in their ST5 proposal and IKAROS by JAXA used reaction control system (RCS) thrusters to spin the sailcraft. The usage of thrusters allows the damping out of any precession or nutation of the spin axis. An attitude change of a spin stabilised spacecraft is usually performed by a forced precession of the spin axis by a series of pulsed thrust actuation's to generate a torque synchronised with the spin rate. In IKAROS this torques are generated by the change of reflectivity of its LCD panels. Bong Wie simulated a 40 x 40 m solar sail with a spin rate of 0.3 to 0.5 deg/s. The pointing error of this sailcraft with an CM/CP offset of 0.1m is about 1 deg.
Reaction wheels
Reaction wheels are internal momentum exchange devices which have been used extensively in the past for fine attitude control. They consist of an electric motor spinning a wheel. Due to the large inertia associated with Solar Sails, large wheels are required which add mass to the sailcraft and thus reducing its efficiency. Wie has carried a study of a 40 x 40 m earth orbiting sailcraft as part of the NMP ST7 mission study. It is concluded that with a cm/cp offset of 0.1m a pitch reaction wheel with a storage capability of within 15 N.m.s is required to counter both the solar-pressure and orbital disturbance torques. Such reaction wheels are in common usage but have a mass of around 6 Kg adding significant mass to the system. This shows that reaction wheels can potentially be used in sailcrafts. If a desired sun angle of 35 deg is required this will cause a reaction wheel momentum growth of 100 N.m.s per orbit causing the reaction wheels to saturate fast. Polities tackles this problem by using magnetorquers to desaturate the reaction wheels. It is shown that a 31 x 31m sailcraft in a 1250 Km circular orbit with a 0.2m cm/cp offset can be controlled using reaction wheels and magnetorquers.
Thrusters
Tip mounted thrusters can be used to control the attitude of a Solar Sail. The placement of the thrusters at the tips of the booms takes advantage of the long moment arm. Fuel lines and cables need to be routed through the booms though which greatly increases the deployment complexity and the boom requirements. Furthermore mass ejection systems due to their dependence on fuel are not suitable for the long duration missions Solar Sails are intended for. Micro Pulsed Plasma Thrusters (PPT) on the other hand though would be more suitable because of their smaller size and power needs. Due to the constant disturbance torques present the PPT would require to be constantly on consuming power. So PPTs in literature are suggested as a secondary/backup system to be used for recovery from off-nominal conditions such as when the sun angle exceeds the primary ACS limits.
CP offset techniques
The techniques listed below are based on a change in the centre of pressure.
Articulated/control vanes
Articulated or control vanes could be described as small movable reflective surfaces usually attached at the tips of the booms. Vanes have been used for a number of years on large asymmetric geostationary satellites for attitude control or to desaturate their reaction wheels. Wie derives the dynamic equation for control vanes utilising four vanes gimballed in two axis for redundancy although he does not provide any control algorithm. Vanes can be used independently to provide full 3-axis attitude control. The vanes need to at least provide a torque twice as large as the expected CM/CP offset disturbance torque. Solar vanes are a good solution for attitude control although they have some of the same shortcomings as thrusters. Primarily since they need to be placed at the tips of the booms for a large moment arm, more risk is added to the already risky deployment.
Sail plane/solar panel displacement
The same principle as in the control vanes is used only this time instead of changing the CP by moving the vanes the whole sail plane is moved. The ends of the solar sail are attached to spars which are mounted on rotatable booms. By rotating the booms a windmill effect is generated controlling the roll of the sail. While the bottom of the sail plane is translated about the roll axis tilting the sail plane controlling the pitch and yaw. Due to the large surface of the sail planes small movements are required. This movement of the sail planes need to be done very slow so as to not excite any vibrations. This system is highly non linear with a large number of indeterminable variables such as wrinkles and bellowing. To alleviate some of this concerns, only a small section of the membrane can be moved.
Another method of control is instead of translating the whole sail plane is to use four bus mounted movable solar panels. This way the attitude control system is decoupled from the sail plane. As discussed earlier the torque generated needs to be twice that of the disturbances. So the large solar panels will be required due to the small moment arm and their low reflectivity. Large solar panels will cause large shadows on the sail plane causing decreased performance and more non linearity. Furthermore the tilting of the solar panels will have an adverse effect on their efficiency for power generation.
Variable reflectivity
IKAROS is the first mission to make use of this new method. It consists of Liquid Crystal Devices (LCD) mounted at the edges of the sail membrane. The reflectivity of the LCD elements is changed thus shifting the CP towards the side with the highest reflection and generating a torque. In the case of IKAROS which is a spin stabilised sail the devices where turned on and off in conjunction with the spin of the solar sail so that compared to the sun, one side is more reflective than the other.
CM offset techniques
Centre of mass (CM) techniques operate much the same way as the centre of pressure techniques, in this case though the CM is offset with respect to the CP. The major advantage of these techniques is that they do not operate on the sail so it is not excited in any way. The disadvantage though is that they might add parasitic mass to the system increasing the sail loading and decreasing the efficiency.
Gimballed control boom/ Gimballed thrust vector control
The gimballed control boom method as the name implies utilises a 2-axis gimballed boom with a mass at the tip. The tip mounted mass can contain instruments or the bus itself. This method was proposed as part of the New Millennium Program Space Technology 7 program. Wie simulates 40 x 40m sail using this technique. He used a state space representation and a linear quadratic regulator to derive the control gains. He has demonstrated the effectiveness of this method in the presence of a CM/CP offset and gravity gradient disturbances although he derive to the conclusion that further study needs to be carried out especially in the presence of mission/hardware constrains. The reaction force due to the movement of the boom produces a torque which actually aids in the attitude change of the sail. A boom though needs to be deployed so as to provide some distance between the mass and the sail to enable movement.
Trim control mass
Another method of changing the centre of mass is using trim control masses. These are essentially small masses running along the length of the booms. This control method was proposed to be used for the Solar Polar Imager mission. Masses of 5Kg which could move 100m were used to control a 160 x 160m 450Kg Solar Sail. A variable limit saturation PID controller was used with success. A different control approach was used by Romagnoli instead of having a feedback controller to complete the manoeuvre a combination of feed-forward and feedback controllers were used. An open loop controller which only uses algebraic equations to determine the state vector of the system and its inputs for the desired manoeuvre was used, complemented by a feedback controller to handle the disturbances. A proportional controller using lead compensation was developed and will be presented in chapter 5. This method though comes with some disadvantages, primarily there is added parasitic mass and also the lanyard system used inside the booms increases the risk of deployment. One method devised to mitigate the risk of deployment is to have separate deployable booms with the masses running though them which are not part of the membrane support. The disadvantage of this method though is, due to the fact that the booms will not be long, in order to achieve the same effect with the smaller moment arm larger larger parasitic masses will be required.
Translation stage
This novel attitude control actuator is essentially a 2-axis table between the sail and the bus. This method solves some of the problems present in the previous methods. It does not add any parasitic mass and does not require any deployable booms. This mechanism is proposed for the CubeSail mission.